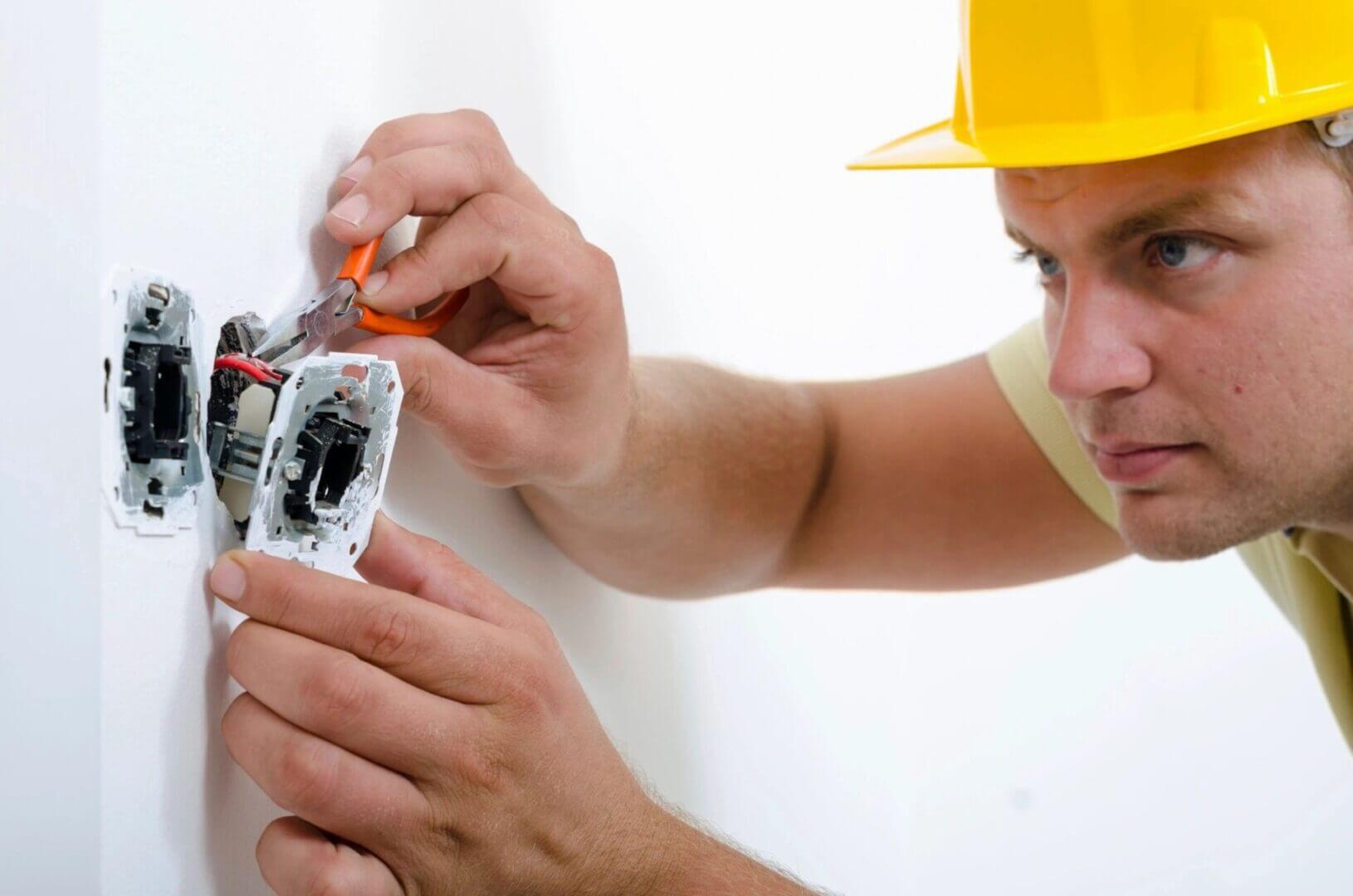
ELECTROCUTION PREVENTION
CETRI recognizes that the most effective treatment for electrical shock and electrocution injuries is to reduce future recurrences. Over the past 20 years, CETRI investigators have contributed to professional standards committee discussions about safe work practice guidelines and protection equipment development in North America, South America, Asia, and Europe. The goal is to continue the refinement of standards based on our evolving understanding of biophysics and the pathophysiology of electrical injury and electrocution.
The following links provide more detailed information.
Additional information can be obtained from the Occupational Safety and Health Association. Call Us here at CETRI if you need additional references.
Other RESOURCES relating to electrical and radiation injuries
A 501 (c) (3) organization dedicated exclusively to promoting electrical safety in the home, school, and workplace.
NFPA provides resources on electrical hazards.
The Center for Disease Control and Prevention provides resources on workplace safety and other prevalent health topics.
AVO consists of a team of instructors, electrical engineers, safety specialists, and support staff committed to eliminating electrical hazards in the workplace and empowering employees to do their work safely and effectively.
An interdisciplinary organization focused on studying lightning and related phenomena to promote lightning safety, prevent injuries, and improve the treatment of survivors.
A not-for-profit support group created by survivors to help survivors, their spouses, and other interested parties.
Dedicated to improving the lives of everyone affected by burn injuries through patient care, education, research, and advocacy.
Offers no-obligation quotes from reliable contractors in Chicago and surrounding Illinois.
A helpline that gives information regarding every form of charitable, long-distance medical air transportation.